Will brain implant technology spark a health revolution?
4 May 2022
Once safely implanted in the brain, a super sensitive sensor will change the lives of families whose children have hydrocephalus.
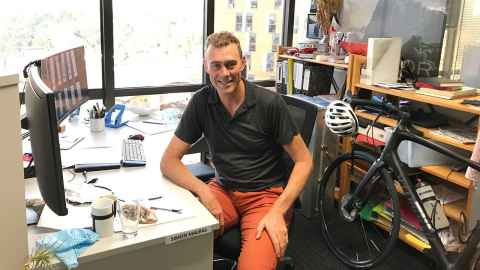
Researchers in the Implantable Devices Group at the Auckland Bioengineering Institute (ABI) are developing a medical pressure sensor to be implanted into the brains of patients with hydrocephalus.
If successful, it would be the first New Zealand-designed fully implanted electronic medical device, akin to the complexity of a cochlear implant or pacemaker. It would alleviate the considerable anxiety and suffering of parents of children with the disorder, as well as highlight the potential of remote monitoring of chronic conditions wherever a patient lives and ultimately, the means of providing more equitable healthcare.
Hydrocephalus is a condition characterised by an increase in fluid around the brain which, unless treated, is fatal. Approximately 100 patients in New Zealand are diagnosed with hydrocephalus every year. Since the 1950s, it has been treated with a thin tube that is surgically implanted in the brain, a shunt, to drain and divert excess fluid away from the brain. The technology has been lifesaving. The problem is that for a range of reasons, the shunt often blocks, resulting in increased pressure around the brain and reduced blood supply to key areas.
“In children, 50 percent of the tubes will have blocked within two years, and they will need further invasive surgery to fix the blockage,” says Professor Simon Malpas, who is leading the team developing the device.
“Imagine being a parent and told your baby has an abnormal build-up of fluid on their brain. You’re grateful that there is a treatment, but then you’re told that the tube inserted into your child’s brain is quite likely to block.”
Another problem is that it is difficult to tell if the shunt is beginning to block, other than by waiting for symptoms to develop. Symptoms of a shunt failing include common ailments such as headaches and nausea, which are common and may or may not be related to the shunt. But that concern is likely to lead to a trip to the hospital (which for many, is a long way from home) followed by a CT or MRI scan of the brain.
The device would offer patients and caregivers both peace of mind and early warning of a likely blockage, thus preventing unnecessary hospitalisations. Bioengineers at the ABI have been working on a solution for several years and have designed and had manufactured a miniature sensor, slightly smaller than a Panadol tablet, that would be placed in the brain alongside and at the same time as the shunt and which would sense if and when pressure in the brain is rising.
Their implantable sensor is part of an intracranial pressure (ICP) measurement system that also includes a handheld ‘wand’ that is held near the head, which ‘wakes up’ the device, and a pressure reading is sent back to the wand. This will typically only need to be used if a patient has symptoms of possible shunt failure but could also be used to detect pressure changes before symptoms occur.
The system enables a measurement of ICP to be read by the patient (or their caregivers) and shared with health care professionals electronically on the Cloud and through a mobile phone, through an app designed by the team. The app would also allow for the collecting of readings over time and symptomatic information. Both neurosurgeons and parents of children with hydrocephalus, have said this would be a “game-changer”.
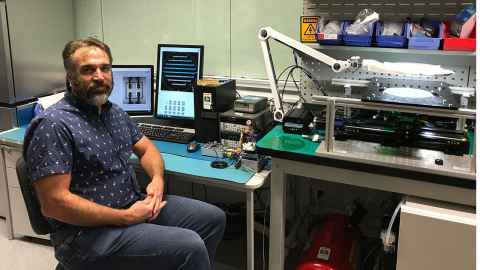
They have developed the ICP measurement system and relationships with manufacturers and are well past proof of concept. However, designing devices is one thing – getting them to market and used in the real world is a longer challenge. It involves identifying the practicalities of manufacturing, and among myriad other prerequisites, preparing the evidence that will be required for regulatory approval.
They will ultimately be seeking approval from the FDA, which recognises three classes of medical devices. Class 1 medical devices are non-invasive and of limited risk to patients or users, such as bandages, crutches, and dental flosses. Class II devices are subject to performance standards, such as blood pressure sensors, thermometers, contact lenses and powered wheelchairs.
Class III medical are typically those of substantial importance in preventing impairment of human health, but also pose the highest risk to patients. Consequently, they are subject to the most rigorous regulatory controls. Examples of Class III devices include implantable pacemakers and automated external defibrillators – and it is expected that this would include a device that could be implanted into the brain of a child with hydrocephalus.
This project has taken the research team through largely uncharted territory. Each of the elements that go into the implantable device have required them to come up with a range of innovations.
Their work has included designing a membrane that will deflect in response to subtle changes of pressure, designing miniature electronic circuitry that will power the device and enable it to gather and send information, and inventing testing equipment to assess the sensitivity and durability of the sensor over time.
At every stage, the team has kept their eyes on the long ‘game’ – the practicalities of getting the device manufactured, into the market and used in the real world. The first stages involved identifying what material it would be made of – that would keep patients, but also the device, safe from, for example, an attack by the immune system.
“While there are numerous sensors on the market that can meet the performance requirements for monitoring brain pressure, none are implantable due to the harsh conditions our bodies subject implanted objects – the body doesn’t welcome foreign objects,” explains Dr Bryon Wright, senior sensor development engineer at the ABI.
“We had to ask ourselves, ‘would we allow our sensor to be put into our own child?’”
We are using the microfabrication techniques developed for silicon chips and using those for glass.
They turned to glass, which Dr Wright describes as “cutting edge packaging” for the sensor. Glass has many virtues. It is biocompatible – a material that is accepted (or, if you prefer, largely unnoticed) by the human body.
“But glass is a very complicated group of materials, and we’re talking about specific types of glass, which have been developed in response to modern microfabrication techniques that are ushering in a new generation or medical implants which have been, until recently, typically made of polymers.”
“We are using the microfabrication techniques developed for silicon chips and using those for glass. This has recently become a hot topic in commercial and industrial applications but using it for medical devices is a developing area, and we're part of that. It represents a next generation material for medical implants.”
The virtue of glass is its quality of hermeticity. In other words, it doesn’t leak – it literally keeps the device hermetically sealed.
“It is a biocompatible material, which means it won’t cause a damaging tissue response in the body, and its hermicity means it won’t leak, both of which reduce risk to the patient and protect the sensitive enclosed electronic components,” says Dr Wright.
“And we’ve have teamed up with world-leading glass manufacturing companies to enable fabrication of glass sensors, ensuring high quality and low costs through scalable production.”
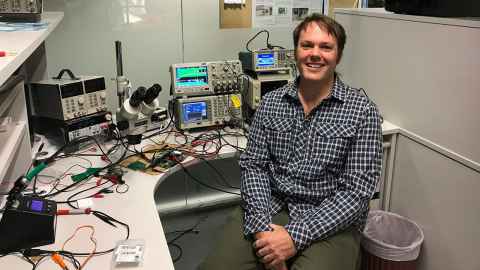
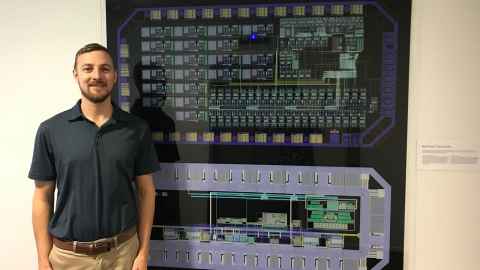
Another key challenge has been finding a way to ‘power’ a miniature device smaller than a Panadol tablet for years and even decades. Drawing on years of research done at the University of Auckland, including the ABI, the team turned to inductive power transfer, or wireless power – thus enabling a power supply for the implant with no battery nor external wires.
“Our group expertise is in powering and communicating with implantable devices, and we have been active in this area for 20 years,” says Dr Daniel McCormick, senior research fellow at the ABI. “This experience has been invaluable in equipping us to develop communication and power transfer systems that can fit in a miniature implantable device.”
There were numerous challenges requiring high-level engineering problem-solving. To cut a complex story short, the team designed a custom-made silicon chip called an application specific integrated circuit (ASIC), a miniature computer that acts as the brain of the implant, incorporates the electronics required to manage power and data transfer and accurately measure pressure.
In other words, they shrunk the electronics. “Miniaturising the electronics is one of the key innovations in this project, that has enabled the development of this technology,” says Dr Robert Gallichan, senior research fellow, whose PhD project focused on using integrated circuits to develop miniature systems for wirelessly transferring power to implantable devices.
“The amount of energy actually going in is very small. It’s very safe for the tissue. It had to be, or it would never get through the regulatory process.”
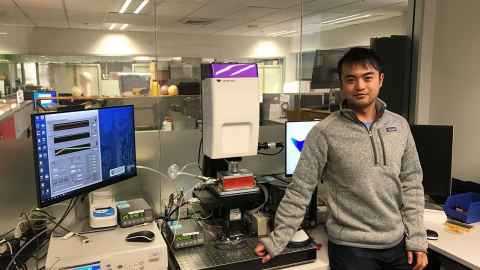
Getting the device to market, which involves gathering the necessary evidence in preparation for getting approval as a life-changing class III medical device was just as challenging.
That involves, prior to clinical trials, physically testing that it works, is safe, and that it will last a long time to ensure patients and physicians can be confident that it will provide accurate measurements for many years.
“We can demonstrate the performance of our sensor with pressure recordings from our test systems,” says research fellow Dr Dixon Leung. He and the team have custom built the systems used to test sensor performance. “We run an array of performance tests to see how our sensors respond when exposed to the physiological conditions found within the brain.”
High-end metrology or weather instruments are incorporated into the test systems. To give a sense of the precision required, the team unintentionally recorded atmospheric pressure changes within their laboratory from the 2022 Hunga Tonga-Hunga Ha’apai volcanic eruption 2000 km away.
“To ensure longevity, we have set trials for our sensors,” adds Dr Leung. “We either provide a long work out, through millions of pressure cycles, or through a ‘relaxing spa’, for weeks, set at body temperature. This is a way of demonstrating the long-term stability of the sensors.”
One of the ways to enhance stability is by studying the surface profile of the sensors. A device called a digital holographic microscope provides video rate 3D scans of surfaces with resolution down to one-thousandth the width of a human hair.
“We observe for physical changes to the sensor surface as it responds to pressure changes. This is a tool to provide feedback as we design a stable sensor."
This is not an academic exercise; we want to make sure this is manufacturable and get it to market. We won’t be satisfied until we can get it into clinics and used in the real world.
The array of innovations to come from their research will contribute to the advance of implantable devices, and the potential of remote and more equitable healthcare.
Says Professor Malpas: “This is not an academic exercise; we want to make sure this is manufacturable and get it to market. We won’t be satisfied until we can get it into clinics and used in the real world.”
“A critical aspect of our project is that we want to transform healthcare from reactive management of a chronic disease to proactive timely care. And offer patients and caregivers’ security that their healthcare needs are being addressed no matter where they live or their socioeconomic status.”
The research and development of the project has been supported by MBIE, the HRC, Cure Kids, the Neurological Foundation, the Auckland Medical Research Foundation, and philanthropic donors.
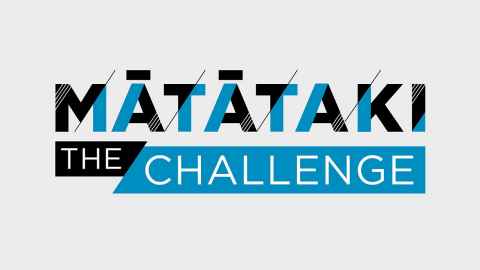
Story: Margo White
Images: Claire Concannon
Mātātaki| The Challenge is a continuing series from the University of Auckland about how our researchers tackle some of the world's biggest challenges.
The Challenge articles are available for republication.
Contact: Gilbert Wong gilbert.wong@auckland.ac.nz